Nuclear fusion occurs in celestial objects, such as the cores of stars, and in thermonuclear weapons. It happens when two light nuclei, such as hydrogen and its isotopes, fuse to produce a larger, heavier nucleus which releases energy. For 50 years, researchers have been trying to mimic this process to generate energy and other national defence applications but building a fusion reactor that can deliver energy in controlled way is not easy.
The Lawrence Livermore National Laboratory (LLNL) in the US recently succeeded in creating a “burning plasma” state at the National Ignition Facility (NIF). The LLNL is one of the two main laboratories in the world working on a technique called inertial confinement fusion (ICF) with high-power lasers1. The other ICF laboratory is the CEA’s Laser Megajoule in France, currently under construction.
Inertial confinement fusion
To achieve thermonuclear fusion in the laboratory, a fuel must be heated to incredibly high temperatures – close to those in the Sun. At such temperatures, the fuel goes from being a solid to a “plasma”, a state in which fusion reactions readily occur.
Once fusion has taken place, more energy must be produced than was put in so that the excess energy can be used in applications such as electricity generation.
There are two main methods to heat and then confine the plasma. The first is “magnetic confinement” in a device called a tokamak. Here, a superconducting ring confines the plasma at relatively low-pressure densities, but at very high temperatures for long periods of time. The second is to use high-power lasers: lasers that emit powerful pulses of light lasting just 10 to 20 nanoseconds that produce between 1 and 2 megajoules (MJ) of energy.
This second technique is known as inertial confinement fusion (ICF) and requires high temperatures and pressures. What is more, once fusion has taken place, more energy must be produced than was put in, so that the excess energy can be used in applications such as electricity generation. The fusion reaction must also be self-sustaining – a process that is triggered by a phenomenon called “ignition”, in which alpha particles that are also emitted during fusion release heat to initiate new fusion.
At the NIF, researchers used a set of powerful lasers focused tightly on a millimetre-sized fuel capsule containing tiny pellets of hydrogen isotopes – deuterium and tritium – suspended inside a cylindrical X‑ray “furnace” called a hohlraum. In this type of experiment, the heat from the X‑rays emitted by the furnace causes the surface of the capsule to explode, or ablate. Thus, by imploding, the surface of the capsule compresses and heats the deuterium-tritium fuel until the hydrogen nuclei fuse into helium, releasing neutrons and other forms of energy.
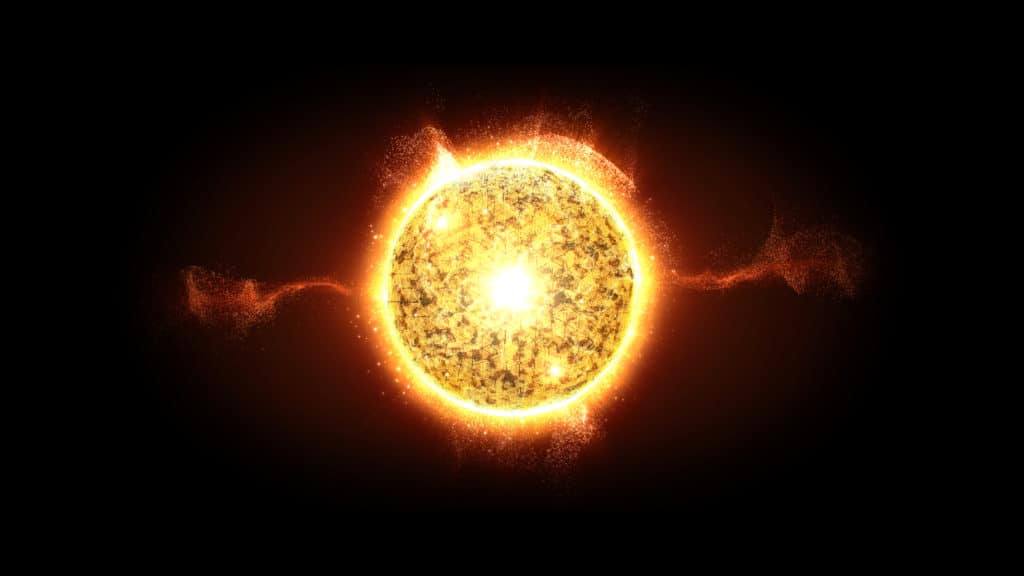
In this type of experiment, we are talking about a capsule that is initially millimetre-sized. We then converge it to a diameter of about 50 microns to increase both the density and the temperature and generate the fusion reaction.
Compressing matter at high speed
Each laser pulse lasts only a few nanoseconds and the lasers can deliver about 1.9 MJ of energy. It is this powerful blast that causes the capsule to rapidly implode, producing extreme temperatures of up to 100 million degrees Celsius. Inside the central hot spot, where the fusion reactions take place, pressure densities are 100 times higher than atmospheric pressure.
The shock created by the laser compresses matter at such high speeds (about 400 km/s) that it reaches enormous kinetic energies. It is only when the compression “stalls” that the kinetic energy is transformed into thermal energy, which is also colossal. Only an instrument such as a high-powered laser has the energy to compress matter in this way.
This is the first time we have a system in which fusion itself provides most of the heat – a key step towards achieving even higher levels of performance. Until now, fusion experiments produced fusion reactions thanks to huge amounts of external heat to heat the plasma.
Will we see ignition soon?
While the NIF has not yet achieved ignition, the researchers have managed to produce 1.35 MJ of energy using 1.9 MJ of laser energy, giving a Q (Efusion/Elaser) of 0.7, where ignition is defined as a Q of 1. We are thus close to the goal.
It is often said that nuclear fusion will still not be feasible in 30 years’ time, but new breakthroughs in this field suggest that – sooner or later – fusion scientists will have the last word.
Lasers at École Polytechnique
At École Polytechnique, there are two lasers, a kilojoule (kJ) nanosecond laser called LULI2000 and the Research Infrastructure (IR*) APOLLON, a potentially multi-petawatt femtosecond laser. The former can be used to produce laboratory plasma conditions close to those associated with inertial confinement fusion, while the latter is intended for fundamental research in the very high intensity regime.
While we cannot experiment with neutron creation because we do not have sufficient input energy or a powerful enough laser beam, we can produce the conditions necessary for fusion – high temperatures and high plasma density – to study the physics of inertial confinement. The plasma we create at LULI will allow us to study plasma microphysics and to test the numerical codes used to design fusion experiments.
LULI operates at micron wavelengths with a maximum energy of about 1 KJ and generates light pulses that last between 10 to 20 nanoseconds. The laser emits a pulse approximately every hour and can be housed into a building at least 80 metres long, which makes it a relatively large experimental facility.